Elements of the past: Big Bang Nucleosynthesis and observation
How to reconstruct the abundances of light elements shortly after the big bang, and thus test some important predictions of the big bang models against observation
An article by Achim Weiss
The theory of nucleosynthesis during the first few minutes after the big bang makes very clear predictions about the abundances of light atomic nuclei in the early universe – about the contributions of hydrogen (single protons), deuterium, helium-3, helium-4, lithium-7 to the total mass of ordinary matter contained within a given region. A brief overview can be found in the spotlight text Big Bang Nucleosynthesis: Cooking up the first light elements. This text, in contrast, is concerned with the crucial question: If we want to test these predictions, how do we determine the original (“primordial”) abundances of the different light nuclei?
Reconstructing the past
Ever since the first stars lit up some three or four hundred million years after the big bang, the abundances of light nuclei have been changing – the universe has undergone what astronomers call chemical evolution: Stars (like our sun) are nuclear fusion reactors – that is how they set free the energy they radiate away in the form of light and other kinds of electromagnetic radiation. Hence, in the interior of stars, light elements are constantly created from even lighter precursors, and destroyed as subsequent fusion produces heavier atomic nuclei. As lighter stars blow off their outer layers to form planetary nebula, or as heavier stars blast most of their substance into space in a supernova explosion, the fusion products are scattered over wide regions of space.
In order to test the predictions of Big Bang Nucleosynthesis, we need to identify astronomical objects in which the original abundance values are preserved as well as possible – and we need to account for any remaining influences of chemical evolution.
Fortunately for astronomers, there are indicators of how much chemical evolution particular objects have undergone, most importantly the presence of elements such as oxygen and nitrogen. These are elements with nuclei that are produced by nuclear fusion reactions in stars, but that definitely could not have been produced during Big Bang Nucleosynthesis. The more oxygen and nitrogen a region of space contains, the more its light element abundances are bound to have been influenced by stellar nuclear fusion.
Helium-4 and Dwarf Galaxies
The most direct – and thus most solid – prediction of Big Bang Nucleosynthesis concerns helium-4, each nucleus of which consists of two protons and two neutrons. However, helium-4 is also a standard product of stellar nuclear fusion.
In order to infer the primordial helium-4 abundance, astronomers turn to certain dwarf galaxies. The following image shows an important example, the galaxy “I Zwicky 18”, a dwarf galaxy rather close to us by intergalactic standards, a mere 45 million light-years away:
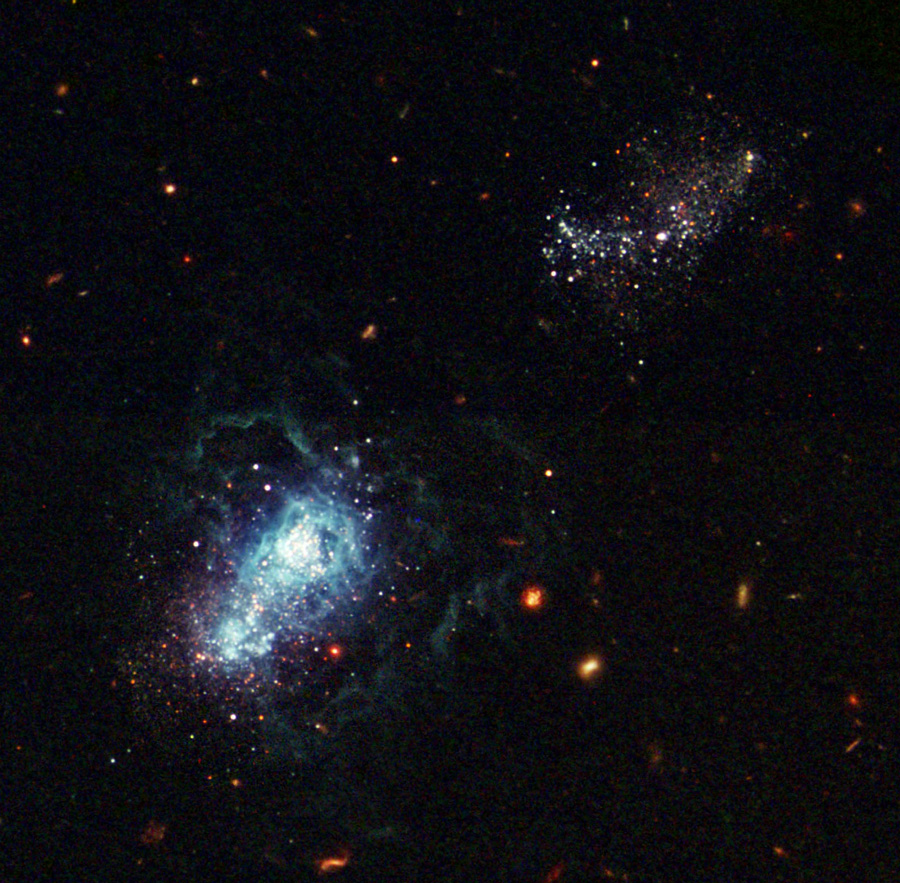
[Image: NASA, ESA, Y. Izotov (Main Astronomical Observatory, Kyiv, UA) and T. Thuan (University of Virginia)]
While there is no direct way to ascertain how much of the helium detected is helium-4, as opposed to helium-3 (the latter has only a single neutron in each nucleus), measurements in our own galaxy show helium-3 to be exceedingly rare, accounting for a mere thousandth of a per cent of total helium. Thus, no significant error is introduced in taking the measured helium abundance to be the same as the helium-4 abundance for a given dwarf galaxy.
When these abundance determinations are performed for a number of clouds and different dwarf galaxies, one can plot the results to show how helium-4 content and oxygen content are related:
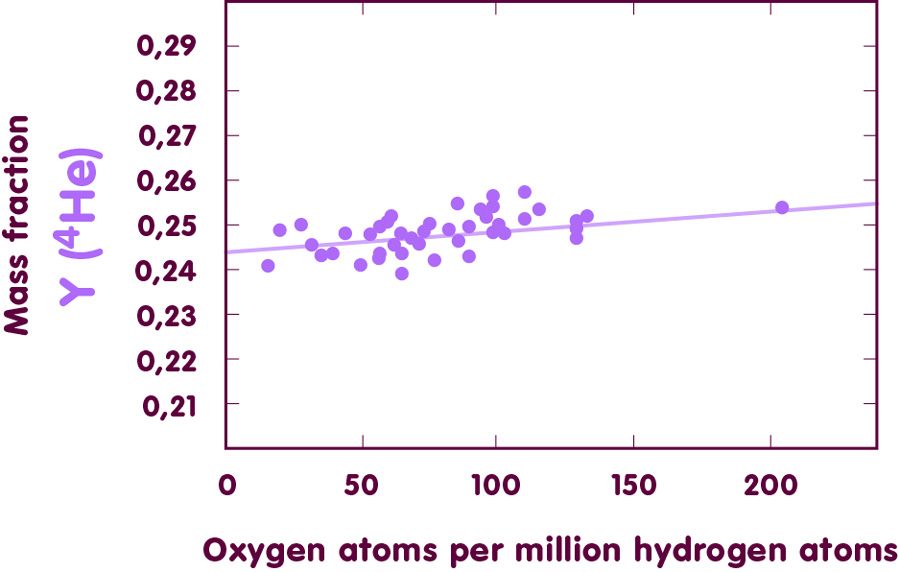
[Image using data from Izotov & Thuan, ApJ 511 (1999) , 639 and ApJ 500 (1998), 188]
All investigations of this type result in a primordial helium-4 mass fraction of 24 per cent, with an uncertainty of little more than one per cent. Not bad for a straightforward extrapolation. In detail, however, there are still disagreements between the different research groups that have performed such evaluations; by a conservative estimate, the true value lies somewhere between 23.2 and 25.8 per cent.
Deuterium and high-redshift quasars
For deuterium (with a nucleus containing one proton and one neutron), another of the light elements produced in Big Bang Nucleosynthesis, the situation is somewhat different. In stellar nuclear fusion processes, any deuterium that might be present- whether primordial or more recent – is quickly converted to helium-3. Consequently, these fusion processes can only destroy, but never produce deuterium. Any deuterium that astronomers can detect must have been produced in the early universe, and any deuterium abundance measured can only serve as a lower limit of the primordial value.
The best current estimates of primordial deuterium abundance make use of some of the oldest objects visible to astronomers: distant quasars, the bright nuclei of active galaxies more than ten billion light-years away from earth. Any light that reaches us from those quasars shows us the universe as it was about ten billion years ago. Analysing the spectrum of the quasars’ radiation, in particular special features (“absorption lines”) due to deuterium and to ordinary hydrogen, the deuterium abundance can be determined. At present, the best results indicate that, in the early universe, there was about one deuterium nucleus for every 30,000 nuclei of hydrogen; more precisely, the ratio of deuterium to hydrogen nuclei was in exponential notation, (3 ± 0.4)·10-5. It is possible that the uncertainty might be higher if, unbeknown to astronomers, there are cosmic clouds between us and the quasars in question, filtering the quasars’ radiation.
Helium-3 and gas clouds within the Milky Way
Helium-3 is probably the most difficult element for which to derive a primordial value. In low-mass stars, it is produced in substantial amounts, while in massive stars, equally substantial amounts of helium-3 are transformed into heavier nuclei. Observations of individual objects are therefore always difficult to interpret.
Also, the properties of helium atoms with helium-3 nuclei and helium-4 nuclei are very similar – the differences are significantly smaller than those between ordinary hydrogen and deuterium. In consequence, it is practically impossible to detect helium-3 in extragalactic systems.
Instead, astronomers turn to our own galaxy. Surveys of all the different elements (including nitrogen and oxygen) show that there is a simple correlation: the closer a region is to the galactic center, the greater the influence of stellar nucleosynthesis on element abundances. In order to estimate the primordial helium-3 abundance, astronomers examine HII-clouds within our own galaxy. While the helium-3 content can only be determined with substantial uncertainty, the results appear to be the same for all regions, whatever their distance to the galactic center.
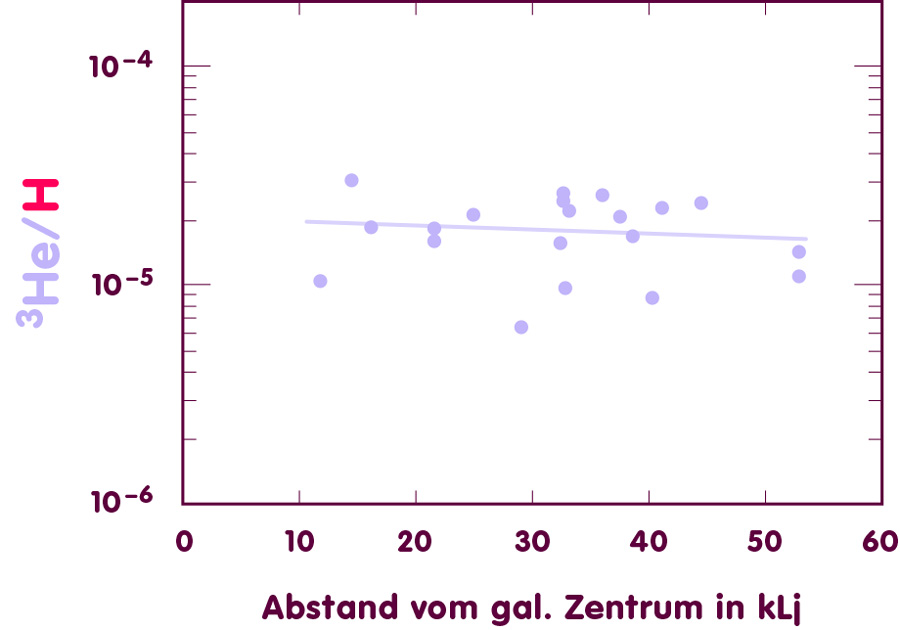
[Image using data from Bania, Rood & Balser, Nature 415 (2002), 54-57]
From the surveys of HII-clouds, one can deduce an average value for the galactic helium-3 content. If we trust the current models to be basically correct in their prediction that on average, more helium-3 is produced in stars than is destroyed, this gives as an upper limit for the original abundance of helium-3. Astronomers have refined this upper limit by looking at an especially well-studied region at a large distance from the galactic centre. In this region, there is one helium-3 nucleus for every 91000 hydrogen nuclei; more precisely, the ratio of helium-3 to hydrogen nuclei is (1.1 ± 0.2)·10-5.
Lithium-7
Finally, and most interesting, there is the abundance of lithium-7 (nuclei with 3 protons and 4 neutrons). These nuclei are produced by nuclear fusion inside some stars, but more commonly they are destroyed. Additional ways of producing lithium-7 must be taken into account: The cosmos is filled with very fast, high-energy particles, mainly protons, which are commonly called “cosmic rays”. When cosmic rays collide with interstellar gas, one of the possible results are lithium-7 nuclei.
Fortunately, it appears that there are some objects in the universe – even in our own galaxy! – in which the original abundance of lithium-7 is preserved: stars that are especially old, and comparatively cool. How do we know?
Stars have a layered structure – nuclear fusion reactions take place in the inner, hotter regions, but not in the outermost layers. Therefore, the composition of the outermost layers should indicate the element abundances for the matter from which a star has formed. For very old stars, that element abundance should be closer to the primordial values. For younger stars, which have formed from material contaminated with the fusion products of stars of previous generations, the abundances will be different.
By systematic analysis of the light received from those outermost layers (more concretely, of the different emission and absorption lines), astronomers can determine the abundances of the layer’s constituent elements. The presence of elements such as oxygen or nitrogen (see above) or, in this particular case, the presence of iron serve as indicators of chemical evolution: Substantial amounts of these elements suggest that a star is young, produced from the debris of other stars. Low abundances indicate that the star is rather old.
For younger stars – recognizable by their high iron content – the lithium-7 abundances can be vastly different, as seen in the following diagram, which plots iron content (horizontal axis; the reference object is our sun) against lithium-7 content (vertical axis; plotted is the number of lithium-7 nuclei per hydrogen nucleus):
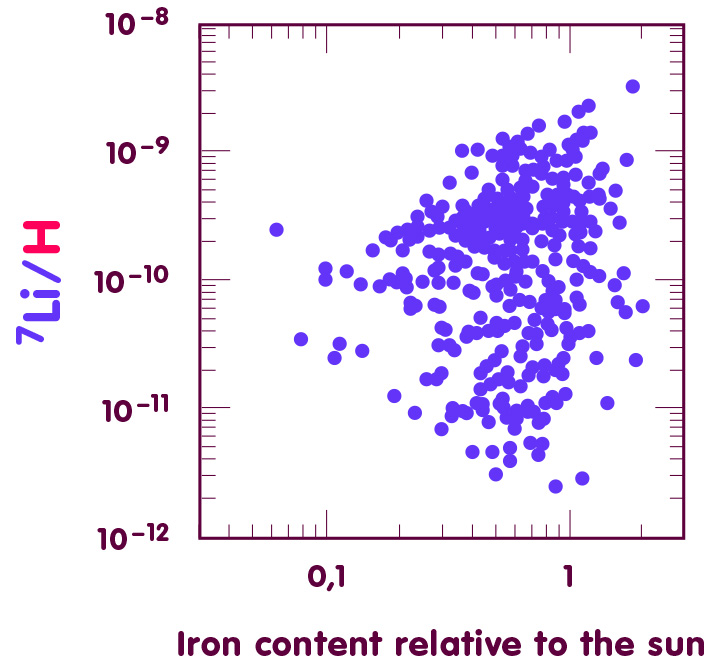
[Image using data from Lambert & Reddy, Monthly Notes of the Royal Astronomical Society 349 (2004), 757]
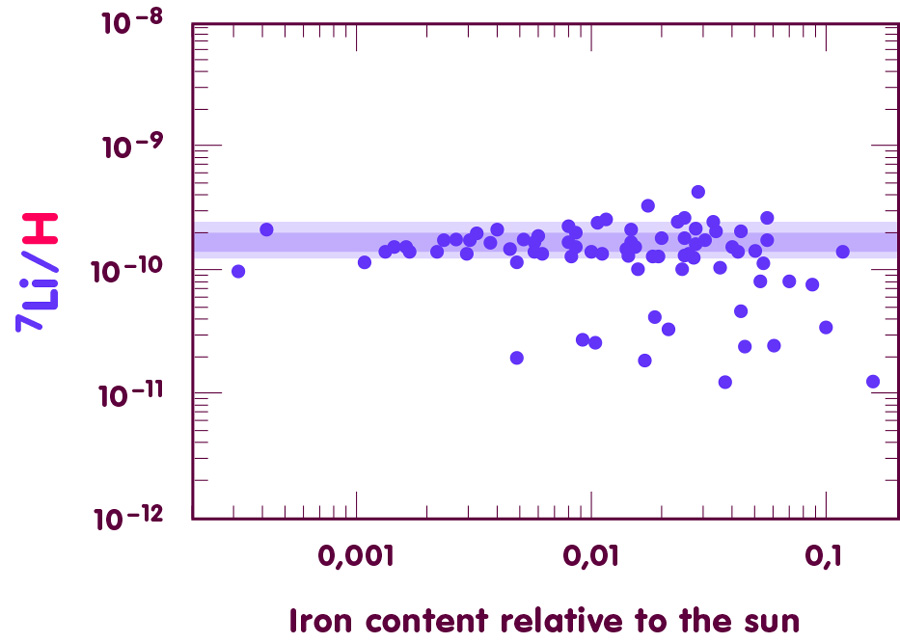
[Image using data from Charbonnel & Primas, Astronomy and Astrophysics 442 (2005), 961]
On average, the stars contain one lithium-7 nucleus for every 8 billion hydrogen nuclei – the ratio of lithium-7 to hydrogen nuclei is somewhere between 1.3·10-10 and 2.0·10-10, although this estimate cannot be expected to be very precise. For instance, elements heavier than hydrogen are expected to migrate slowly towards deeper layers of the star, a cosmic analogue to the more familiar phenomenon of sedimentation: if you let a mixture of water and sand settle, the sand will collect at the bottom, following the earth’s gravitational pull.
Putting it all together
Armed with the results described above, we can confront the predictions of Big Bang Nucleosynthesis with astronomical observation. It is usual to plot the predictions against a parameter denoted by the greek letter eta, which is defined as the total number of protons and neutrons in our universe, divided by the number of photons in the cosmic background radiation. Here, we will concentrate on a narrow range of eta values. A more general overview, covering a much wider range of eta, can be found in the spotlight text Big Bang Nucleosynthesis: Cooking up the first light elements.
Let us start with helium-4:
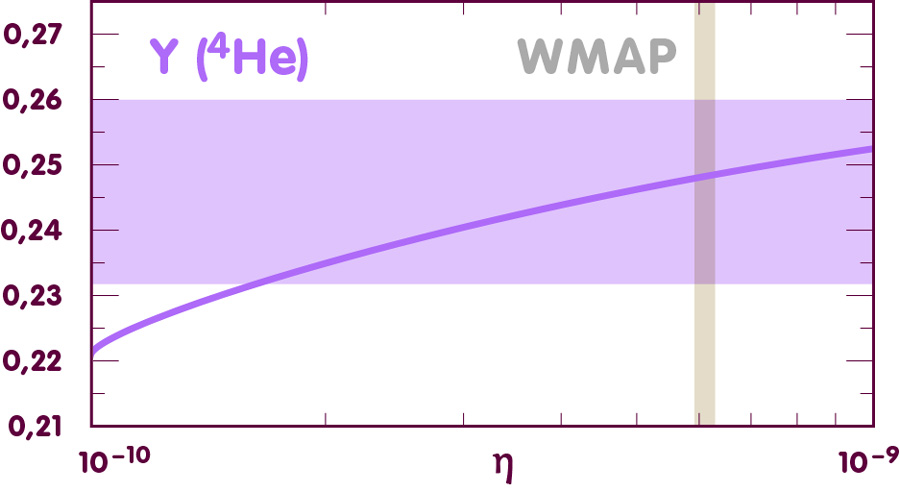
[Image using data from E. Vangioni, Institut d’Astrophysique de Paris]
In this diagram, the vertical axis represents the helium-4 abundance – for instance, a value 0.25 indicates that helium-4 nuclei contribute 25% of the total mass of all atomic nuclei. The curve indicates the theoretical prediction for this abundance.
As the diagram indicates, the conservative estimate derived from the observations covers a very large range of possible values for eta, which is consistent both with the prediction and with the WMAP determination of eta.
The next diagram shows the results for helium-3 and deuterium:
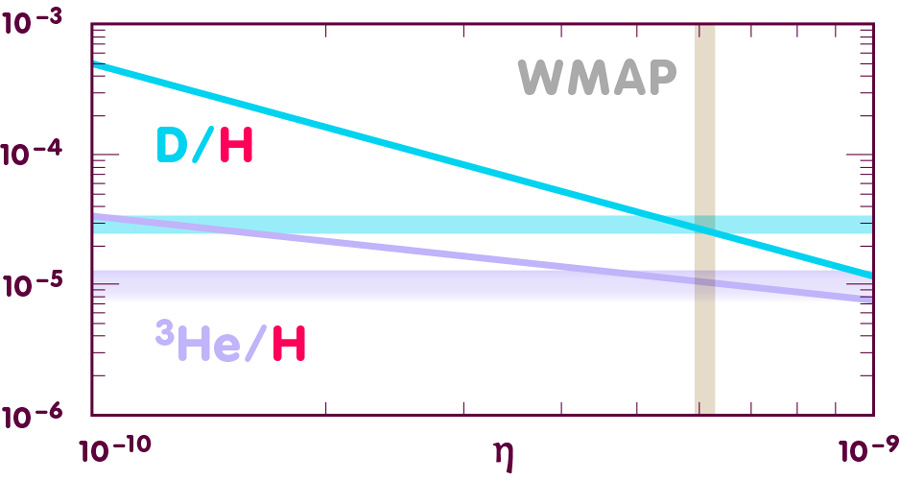
[Image using data from E. Vangioni, Institut d’Astrophysique de Paris]
More problematic is the lithium-7. The following diagram shows the prediction and observational results for the numerical abundance:
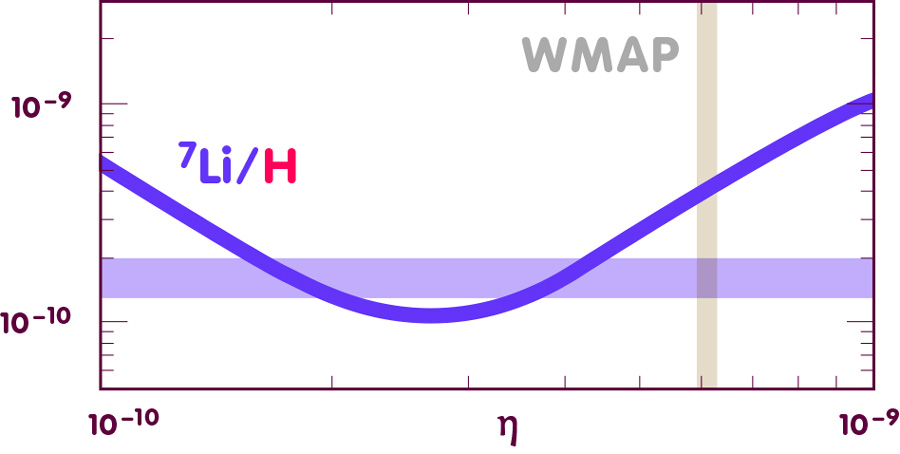
[Image using data from E. Vangioni, Institut d’Astrophysique de Paris]
Overall, Big Bang Nucleosynthesis is strongly supported by observations. But for lithium-7 and, incidentally, for the rather uncertain values for helium-3, much work will be needed to determine the primordial values from the observed data with greater accuracy. As this work progresses, we are much more likely to learn about stellar physics than about the early universe.
Further Information
For the relativistic ideas behind this spotlight topic, check out Elementary Einstein, especially the chapter Cosmology.
An overview of Big Bang Nucleosynthesis can be found in the spotlight text Big Bang Nucleosynthesis: Cooking up the first light elements; information about the physics behind the predictions in Equilibrium and change. Other related spotlights on relativity can be found in the section Cosmology.
References
The theoretical predictions in the diagrams above were kindly provided by E. Vangioni, Institut d’Astrophysique de Paris (private communication, 2005); for further information about these calculations, see
Coc, A. et al., Astrophysical Journal 600 (2004), p. 544 [available online as e-print astro-ph/0309480]
The observational values quoted above were taken from the articles listed below. As both the predictions and the analyses of observations are bound to improve over time, some changes are to be expected.
Helium-4: Olive, K. A. & E. A. Skillman, Astrophysical Journal 617 (2004), p. 29 [available online as e-print astro-ph/0405588]
Helium-3: Bania, T. M., R. T. Rood & D. S. Balser, Nature 415 (2002), p. 54.
Deuterium: O’Meara, J.M., et al., Astrophysical Journal 552 (2001), p. 718 [available online as e-print astro-ph/0011179]
Lithium-7: Charbonnel, C. & F. Primas, Astronomy & Astrophysics 442 (2005), p. 961 [available online as e-print astro-ph/0505247]
Colophon
Achim Weiss is a scientist at the Max Planck Institute for Astrophysics in Garching near Munich, in Germany. His main area of research is stellar physics.
Citation
Cite this article as:
Achim Weiss, “Elements of the past: Big Bang Nucleosynthesis and observation” in: Einstein Online Band 02 (2006), 02-1019