Multi-messenger astrophysics and numerical relativity
During neutron star mergers, both gravitational wave and electromagnetic signals are emitted. This could help solve long-standing issues in basic physics research with multi-messenger astrophysics.
An article by Masaru Shibata
The first detection of gravitational waves from a binary neutron star merger on August 17th, 2017, marked the beginning of a new era in astrophysics. Not only had the LIGO observatories recorded gravitational wave signals from such an event, but researchers around the world also observed its electromagnetic counterparts. This breakthrough announced the beginning of multi-messenger astrophysics.
The merger of neutron star binaries (neutron star and neutron star or black hole and neutron star) is one of the most interesting and important observational targets in multi-messenger astrophysics. They represent cases of very strong gravity, which still aren’t fully understood, and that makes them interesting enough already. On top of that, the study of neutron-star mergers could help scientists to solve long-standing issues in basic research in physics.
What neutron star mergers reveal about long-standing physics questions
One of the open questions is the nature of the high-density nuclear matter. Neutron stars are an ultra high-density object composed primarily of neutrons, as the name suggests. However, their exact composition is unknown. Gravitational-wave and electromagnetic signals from neutron star binaries can provide information on the radius of neutron stars, which is determined by their composition.
Another long-standing issue in physics is the origin of heavy elements like gold and uranium. We believe that these heavy elements are synthesized through the rapid neutron capture process (so-called r-process) from relatively light elements like iron. For this process to occur, an extremely neutron-rich (i.e. proton-poor) environment is necessary – which can only be found in a relativistic setting. However, exactly which astrophysical phenomenon is the major site of this r-process nucleosynthesis is still unknown. Neutron star mergers are the most promising candidates, because they provide a very neutron-rich environment. During a neutron star merger, a fraction of the neutron-rich matter is explosively ejected from the system into the interstellar space. In the ejecta, heavy elements are supposed to be synthesized via the r-process nucleosynthesis. The initially synthesized heavy elements are very neutron-rich, and thus strongly radioactive. This causes them to subsequently decay, which heats up the ejecta and makes them shine. If one can observe high-luminosity ejecta with an electromagnetic telescope together with gravitational waves, one can verify that the r-process nucleosynthesis occurs in the neutron-star merger.
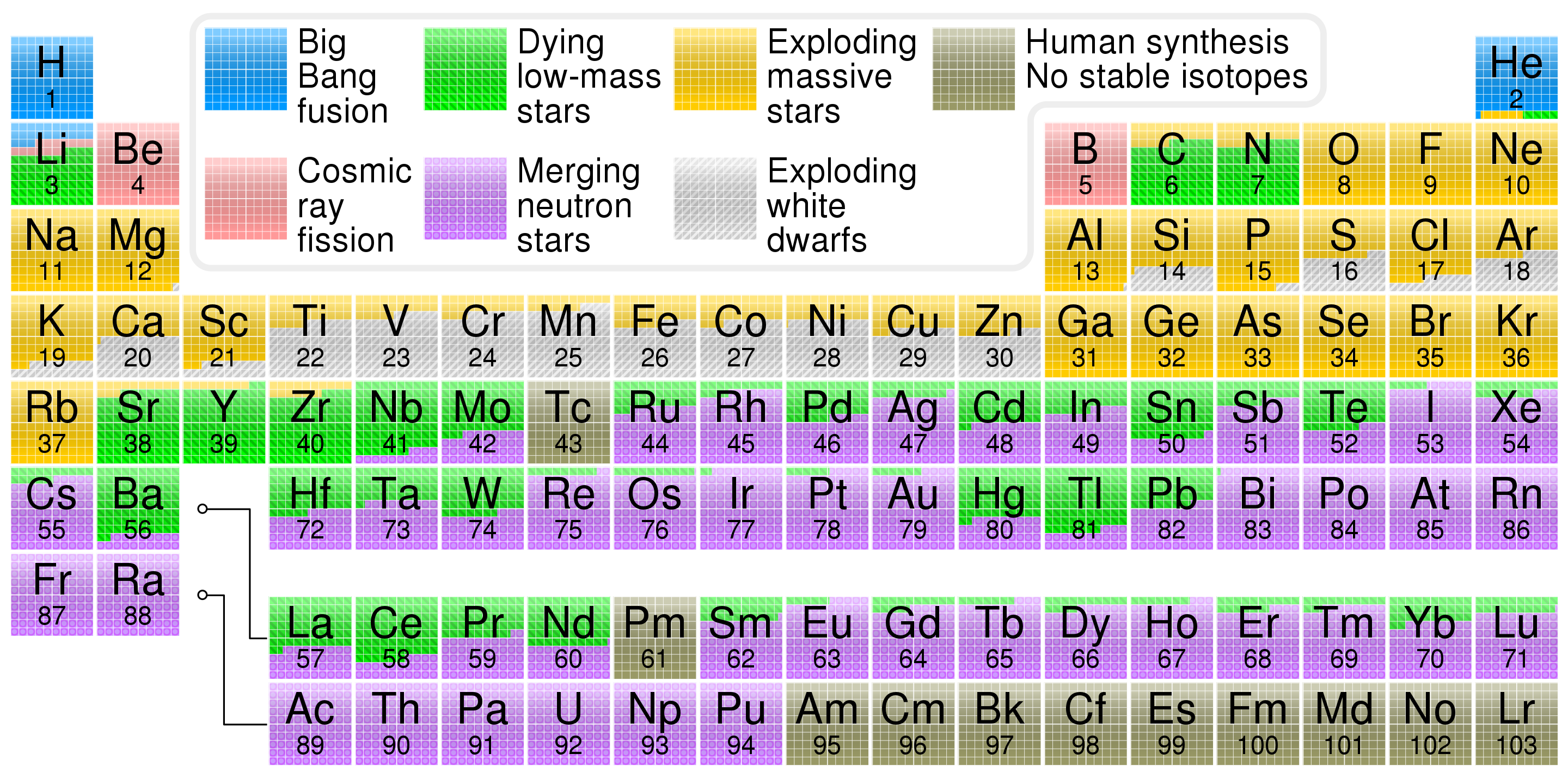
Periodic table showing origin of elements in the Solar System, based on data by Jennifer Johnson at Ohio State University. © Cmglee, CC BY-SA 3.0, https://commons.wikimedia.org/w/index.php?curid=31761437
Multi-messenger observations and numerical relativity
Multi-messenger observation of neutron star mergers could thus help answer a number of long-standing open questions in physics, provided that we have a reliable theoretical model that describes this type of events, with their strong gravity regime and mass ejection. Theorists have to solve Einstein’s equation for general relativity together with the associated matter equations like hydrodynamics equations and radiation transfer equations, which govern the evolution of neutron star matter and neutrino emission. Since these equations are highly nonlinear, multidimensional, and complicated, they can only be solved with a large-scale numerical simulation.
Findings of numerical relativity on neutron star mergers
One important finding of numerical relativity concerns the nature of the remnant after a merger: If the total mass of the binary system is smaller than about 2.8 solar masses, a neutron star is formed. If the total mass of the binary system is larger than this threshold, the self gravity of the merger remnant cannot be sustained by the pressure associated primarily with the repulsive force among nucleons and centrifugal force by rapid rotation of the merger remnant. As a consequence, the remnant collapses to form a black hole.
In addition, numerical relativity has clarified that during and after the merger, a fraction of the matter is ejected. It accumulates in a large disk around the system, irrespective of the type of remnant. The simulations allow to identify two mechanisms of this mass ejection. Dynamical mass ejection occurs in less than 10 milliseconds after the onset of the merger. The matter ejected in this process is called “merger ejecta”. Post-merger mass ejection occurs from the disk surrounding the central object. The time scale of this mass ejection is a tenth of a second to ten seconds, and the ejected matter is called “post-merger ejecta”.
Current state-of-the-art numerical relativity enables scientists to accurately predict the merger and mass ejection processes for a given neutron-star binary (see, e.g, a visualization of a binary neutron star below). Furthermore, astrophysicists can calculate an electromagnetic signal from the ejecta via a radiation transfer simulation, where the energy transport by photons is taken into account. This theoretical work is used to predict signals of gravitational and electromagnetic waves, and serves in the interpretation of the observational results.
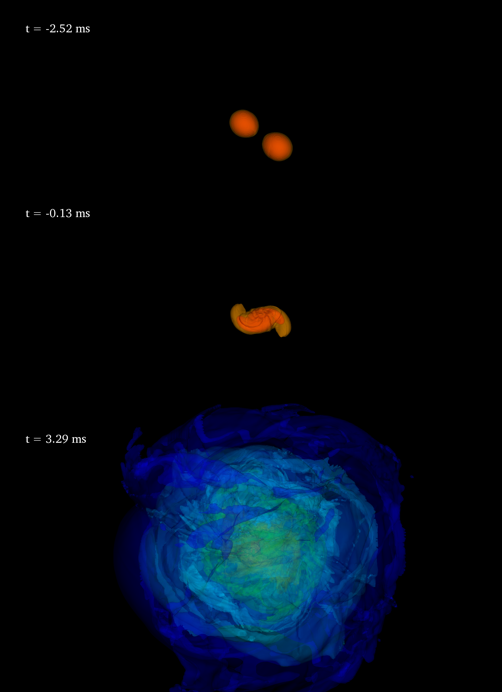
A result of numerical-relativity simulation for the late inspiral (top), merger (middle), and post-merger (bottom) of a binary neutron star. In this example, after the merger, a massive neutron star is formed and a fraction of matter is ejected. © S. Fujibayashi, M. Shibata (Max-Planck-Institute for Gravitational Physics)
Numerical relativity meets real observations
For the neutron-star merger event on August 17, 2017, electromagnetic observations were extensively performed from the gamma-ray to the radio bands. Gravitational wave observation revealed that the total mass of the binary neutron star was 2.73–2.78 solar masses, which is smaller than the 2.8 threshold for black-hole formation. Thus, according to the simulations, the remnant should be a high-mass neutron star. Based on the numerical relativity study, a schematic picture of the merger remnant and ejecta at about one second after the onset of the merger for GW170817 event becomes evident: A massive neutron star at the center is surrounded by a disk. In the outer region, merger ejecta and post-merger ejecta escape from the system with sub-relativistic speed of 5 to 30 percent of the speed of light.
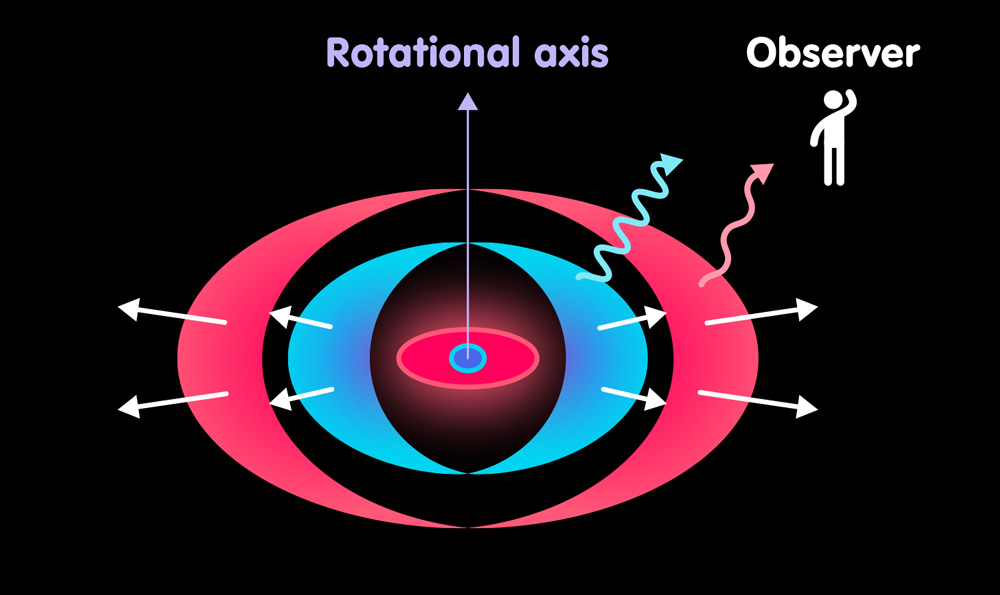
Schematic picture for the remnant of GW170817 at about 1 second after the onset of merger.
Based on these findings, it is possible to construct an ejecta model of a realistic density profile, velocity profile, composition, and temperature. In the case of GW170817, the observed optical and infrared light curves matched the theoretical calculations reasonably well for a variety of wavelengths. The estimated total ejecta mass was about 0.03 solar masses and in addition, it is strongly suggested that in the merger ejecta, a substantial fraction of heavy elements are included.
During the third observation run of the gravitational wave detectors LIGO and Virgo, gravitational waves from what is most likely to be another neutron star merger were detected. Despite extensive immediate search triggered by a public alert system, no electromagnetic counterpart of GW190425 was found. Thus, the multi-messenger observation of a binary neutron star in GW170817 continues to be the primary example for a fruitful cooperation between experimental gravitational-wave physicists, electromagnetic astronomers, and numerical relativity researchers.
Further Information
References
A. P Abbott et al., Phys. Rev. Lett. 119, 161101 (2017).
M. Shibata, “Numerical Relativity” chapter 8 (world scientific, 2016).
M. Shibata and K. Hotokezaka, Ann. Rev. Nucl. Part. Phys. 69 (2019).
M. Shibata et al., Phys. Rev. D 96, 123012 (2017).
K. Kawaguchi, M. Shibata, and M. Tanaka, Astrophys. J. 865, L21 (2018).
Colophon
Masaru Shibata is director of the department Computational Relativistic Astrophysics at the Max Planck Institute for Gravitational Physics in Potsdam and professor at the Yukawa Institute for Theoretical Physics, Kyoto University, Japan.
Citation
Cite this article as:
Masaru Shibata, “Multi-messenger astrophysics and numerical relativity” in: Einstein Online Band 13 (2021), 1011